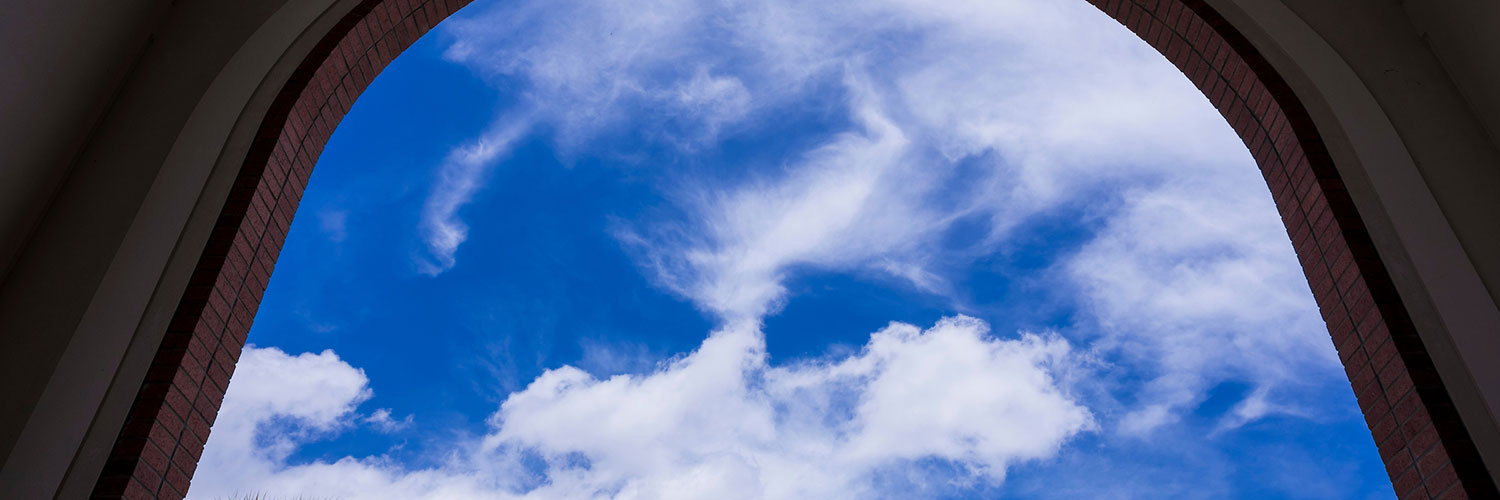
Warren, Donald
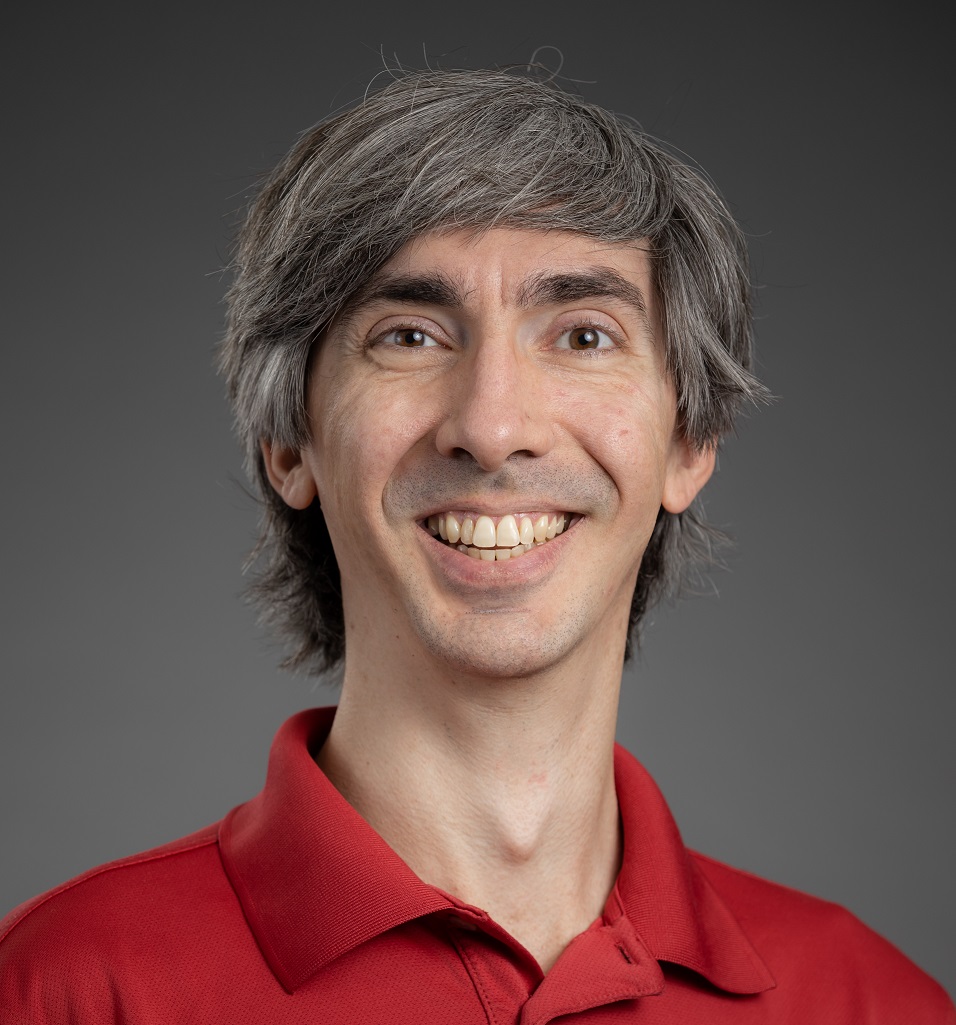
Donald Cameron Warren
Assistant Professor | College of Engineering and Science - Aerospace, Physics and Space Sciences
Contact Information
OPS 335
Personal Overview
I am a computational astrophysicist studying high-energy phenomena:
- Supernovae & their remnants
- Gamma-ray bursts & their afterglows
- Cosmic rays and acceleration processes
In addition to those areas, I have also done work in virophysics and in using virtual reality as a tool for scientific visualization.
When I'm not working (a state that is increasingly uncommon lately), I enjoy watching sports and playing board games. Prior to joining the faculty at Florida Tech, I lived for several years in Japan. I won't say which country (US or Japan) is better overall, but I will say that the Tex-Mex food is better here and the sushi is better there.
Educational Background
2002-2006: BS Physics, BS Mathematics from NC State University
2008-2015: PhD Physics from NC State University
Professional Experience
2018-2022: Research Scientist, Interdisciplinary Theoretical and Mathematical Sciences Program (iTHEMS), RIKEN, Japan
2015-2018: Foreign Postdoctoral Researcher, Astrophysical Big Bang Laboratory, RIKEN, Japan
Current Courses
Spring 2023: SPS 3030 Orbital Mechanics
Research
Supernovae & supernova remnants (SNRs)
Beyond their intrinsic cool factor as "explosive deaths of entire stars", supernovae are terribly important to astrophysics. Among other things, they seeded the Universe with elements heavier than lithium (without which you would not be reading this right now), and they are currently used as probes of the history of the Universe on its largest scales.
However, it is both technologically challenging and somewhat dangerous to explode a star in a terrestrial laboratory. We must look to the skies to study supernovae in order to answer the questions of what makes them happen, how they proceed once they are triggered, and how they evolve over longer times.
Unfortunately for astronomers and astrophysicists (but fortunately for virtually all other life on Earth), supernovae are usually too far away to appear as anything other than point sources of light. If we want to use the 3-D structure of these events to infer physical mechanics, we have to look closer to home. This means using the remnants of historical (and in some cases prehistoric) supernovae: the structures left over hundreds or thousands of years after the supernova, caused by the ejecta crashing into the material surrounding what used to be a star or stellar system.
My work has studied the link between supernova remnants (SNRs) and the initial conditions within the supernova. The chief question we wanted to answer was "Is it possible to work backwards in time, and use the current state of the SNR to inform us about the first seconds or minutes of the supernova?" If that sounds about as challenging as using a driver's license photo to study early childhood conditions, well, that's why we scientists find it exciting!
Gamma-ray bursts (GRBs) & afterglows
Supernovae are caused by exploding stars, and are routinely detected at such great distances the light took a billion years or more to get from the host galaxy to Earth. Gamma-ray bursts are their bigger, badder cousin. In some supernovae, the center of the star creates a jet of matter and energy moving at almost the speed of light. That jet drives through the entire star and explodes out into the Universe as a highly-focused, extremely intense, beam of gamma-ray light. If you lie within the beam, you see the namesake burst of gamma rays. If the beam points away from you, you see almost nothing.
The conditions within a GRB are extreme, and far beyond anything accessible to experiment on Earth. So studying GRBs and their later evolution allows us to explore how physics happens in environments we sincerely hope never occur on or near Earth.
My primary area of focus within this topic is the afterglow of the GRB. After the burst of gamma-rays fades, the jet is still present, expanding outward from the central engine and wreaking havoc in the local neighborhood. That jet produces light at all wavelengths, from radio up to even higher-energy gamma-rays, that we can sometimes detect at Earth. By comparing models for GRBs against the observations, we support or rule out models and slowly work our way towards a more complete understanding of what takes place in these extraordinarily violent settings.
Cosmic rays (CRs) & acceleration processes
Cosmic rays are subatomic particles (electrons, protons, or heavier nuclei) that have been given large amounts of energy. This bland, if correct, definition omits a lot of what makes cosmic rays so exciting to study. The single highest-energy particle ever detected at Earth was a cosmic ray with macroscopic amounts of energy: the so-called "Oh My God" particle -- a single proton or atomic nucleus -- had as much energy as a baseball thrown by a professional pitcher. When high-energy cosmic rays hit the Earth's atmosphere, they create cascades of particles detecable across many square kilometers of Earth's surface. They carry enough energy to produce antimatter (in small amounts, mind you). And we don't know where they come from.
The spectrum of CRs that we detect at Earth extends over more than ten orders of magnitude in energy. At the lowest end, we know that shock waves in the Solar System can donate energy to electrons and protons to turn them into cosmic rays. At higher energies, you need correspondingly more energetic sources. Scientists think that supernovae and their remnants are responsible for cosmic rays up to the so-called "knee" -- a break in the spectrum occurring at about a PeV in energy. (For comparison, the Large Hadron Collider tops out at 0.01 PeV.) But the cosmic ray spectrum carries on for another factor of a million in energy beyond that. Such energetic cosmic rays almost surely come from outside the Milky Way, but that makes the task of identifying their origin all the more challenging. Scientists have considered GRBs, supermassive black holes, and even colliding galaxy clusters as the sources for such ultra-high energy CRs (UHECRs).
My work here has studied the diffusive shock acceleration process -- the leading candidate for how astrophysical sources can transfer energy into particles and transform them into cosmic rays. Do the accelerated cosmic rays interact with the shock and modify the acceleration? (The answer is yes, they should.) How does shock speed affect the acceleration process and the nonlinear interaction between CRs and shock? How does the specific particle being accelerated -- electron vs proton, or helium nucleus -- affect the resultant spectrum? Do we even have the right models to discuss how particles move in the vicinity of an energetic astrophysical shock?
I am also affiliated with the Telescope Array, a CR observatory covering thousands of square kilometers in the deserts of Utah. With such a large observing area, the TA routinely detects UHECRs, and in such quantities that we can do meaningful science with them.
Virophysics
My work in virophysics started from a simple question: how do you count viruses? It would be awkward to put a bunch of viruses under a microscope and shift them from one pile to another, and all the methods for counting them in bulk have drawbacks and limitations. It is nonetheless important to have a good idea of viral count if, for example, you wanted to study the effectiveness of an antiviral drug, or if you wanted to measure the viral load of a sample at various times.
In the end, my collaborators and I developed a tool to take the standard viral assay and compute the viral concentration in a way that is both robust and accurate despite noisy data.
Virtual reality (VR) for scientific visualization and outreach
The Universe as we experience it contains three spatial dimensions, but almost all of us use two-dimensional objects -- paper, or screens of some variety -- to communicate. Having access to the third dimension can be extremely useful for understanding scientific data, but it is not possible when you are limited to a 2-D surface. Virtual reality places the user in a 3-D environment where such data can be presented in complete fidelity, taking advantage of our brain's natural ability to make sense of the world.
In addition to being useful for scientists to visualize their own work, VR is a great way to build interest from the non-science public. It is one thing to talk about how a supernova explodes, and another thing to show someone a video of a simulation. It is a viscerally different experience to place them next to such an explosion and have them standing inside the supernova after it sweeps them up. Such an encounter is not possible using only screens, but it is exactly the use case VR was designed for.